Biotekinstruments.com.br
3D Cell Culture: A Review of Current Techniques
Brad Larson, Senior Applications Scientist, Applications Department, BioTek Instruments, Inc., Winooski, VT
Over the last decade, a central focus of drug discovery efforts has been the incorporation of
in vitro testing models that better mimic
in vivo conditions found within the target patient. An initial step saw a move away from biochemical assays using purified drug target, in favor of a cell-based approach which utilized over-expression of drug target in common host cell lines, such as CHO and HEK-293. The quest for greater physiological relevance proceeded to the use of primary cells, preferably human if supply was adequate, and the reliance on endogenous expression of drug target should detection technology be sensitive enough. A large percentage of these cell types, being naturally adherent, allowed simple culturing workflows that seeded cells in a coated microplate well, incubating the microplate to encourage the cells to attach in a two dimensional (2D) monolayer before performing the prescribed assay. While providing initial improvements over biochemical and immortalized cell lines, an abundance of evidence now supports the reality that culturing cells in this 2D manner is often problematic and is a relatively poor model for
in vivo conditions and behaviors. Using a 2D model, attrition rates of drug candidates for cancer were approximately 95%1, stemming from
in vitro drug efficacy values that did not translate to the clinic, as well as unforeseen toxicity issues. In 2011 alone, out of approximately 900 anti-cancer therapies in clinical trials or under Federal Drug Administration review2, only twelve achieved approval3; resulting in the loss of hundreds of millions of dollars that were spent on pre-clinical and clinical trials. The reason for these shortfalls can be traced to using conventional 2D conditions, where extracellular matrix (ECM) components, cell-to-cell and cell-to-matrix interactions, important for differentiation, proliferation and cellular functions
in vivo, are lost4.
Figure 1. Tumor Microenvironment5.
Parallel research also indicates that traditional 2D cell culture methods may not accurately mimic the 3D
in vivo environment in which cancer cells reside (Figure 1), as the 2D environment does not allow
BioTek Instruments, Inc.
for areas of hypoxia, heterogeneous cell populations (including stromal cells), varying cell proliferation
P.O. Box 998, Highland Park,
Winooski, Vermont 05404-0998 USA
zones (quiescent vs. replicating), ECM influences, soluble signal gradients, and differential nutrient and
Phone: 888-451-5171
metabolic waste transport6 (Figure 2). As a result, the unnatural 2D environment may provide inaccurate
Outside the USA: 802-655-4740
data regarding the predicted response of cancer cells to chemotherapeutics7.
Email:
[email protected]
www.biotek.com
Copyright 2015
White Paper
Figure 2. Schematic of three microenvironmental regions in a centrally necrotic tumor. A
spontaneous tumor may consist of many such necrotic foci. Decreasing magnitude of various
physiological parameters is indicated as +++, ++, +, +/-, and -6.
Additional studies demonstrate that individual drug targets may not be expressed, or the level of cell signaling may not be equivalent to that found
in vivo, thereby impacting experimental results. Indeed, a research study demonstrated that in melanoma cells, 106 genes were up-regulated and 73 genes down-regulated using tumor-like models compared to baseline expression of 2D monolayer cell cultures of the same cells8 . What is interesting is the fact that the genes found to be up-regulated in the 3D model were also found to be up-regulated in tumors.
Many of the same concerns with using 2D cell culture to create accurate tumor models extend to liver toxicity studies as well. While the gold standard for xenobiotic toxicity testing involves
in vivo animal studies, increasing animal welfare concerns, as well as the poor concordance of animal study results to disease phenotypes observed in heterogeneous human populations, make incorporation of a workable, predictive
in vitro testing method a priority9,10. While immortalized liver-derived cell lines simplify procedures and eliminate the need for whole animal testing, the expression profile of genes involved in phase I and phase II metabolism do not correlate well to that observed in liver tissue11. Primary hepatocyte cultures provide levels of functionality much closer to that seen
in vivo, but these cells are problematic when used
in vitro. Under traditional 2D culture conditions, the cells de-differentiate, rapidly decrease expression of cytochrome P450 enzymes, and eventually lose viability12.
The wealth of research highlighting the limitations of 2D cell culture, both as
in vivo tumor and liver models, highlights the need for new cell models in research methods. This demand can be met through the adoption of 3D cell models, as 3D cultured cells exhibit features that are closer to complex
in vivo conditions13. Advantages of incorporating 3D cultured cells, compared to 2D culture models, for evaluation of drug candidates can include: (1) oxygen and nutrient gradients, (2) increased cell-to-cell and cell-to-ECM interactions, (3) non-uniform exposure of cells within a 3D structure to the test molecule, (4) varying cell proliferation zones, and (5) the impact of site specific stromal cells in the tumor microenvironment5. Studies show that tumor cells of specific cell lines, when evaluated in a 3D format, are less sensitive to anti-cancer agents than when the same cells are cultured in 2D formats14. However, other research shows that different cell lines, using a different 3D technology, demonstrate the opposite effect15. These findings highlight how the use of 3D cell culture in cancer research may provide key insights into drug activity
in vivo that may be overlooked if limited to 2D cell culture models only. In addition, the mechanisms involved to create these differences can be elucidated, such as signaling pathway variations, or a shift in the dependence on the target in a 3D system compared to cells cultured using 2D methods.
When cells are grown in basement membrane-like gels, there is a mutual integration of the signaling pathways16. A549 3D spheroids demonstrate constantly high levels of IL-6 and IL-8 secretion when compared with their monolayer counterparts. Enhanced extracellular matrix deposition for better biomarker expression was reported using 3D culture systems17. The differentiation of mesenchymal stromal cells to chondrocytes using hyaluronic acid (HA) 3D model was also analyzed. It was found that the cell receptors could interact with HA better and influence cell differentiation. Various factors, including biologically functional microenvironment, material chemistry, cellular interactions, and mechanical property enhanced chondrogenesis18.
Similar findings to those seen with 3D tumor models are also observed when incorporating 3D cell cultures in hepatotoxicity studies. Wu et al. observed that 3D cultured rat hepatocytes maintain a more differentiated state as compared to a monolayer culture19. Rapid decreases in CYP1A2 and -1A1 expression using traditional 2D monolayer
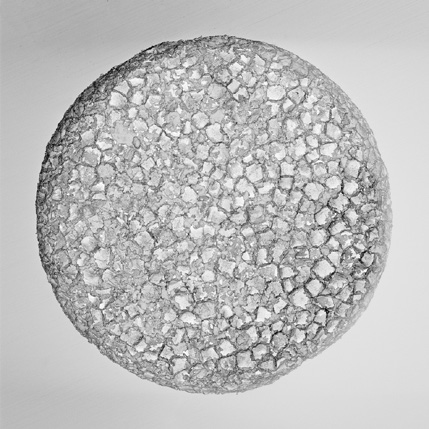
White Paper
culture of mouse hepatocytes, compared to consistently high levels using a 3D model were also detected over a five day incubation period20. Longer-term evaluations were performed by Kratschmar et al., with the evaluation of rat hepatocytes maintained for 25 days using either a 2D sandwich culture or 3D co-culture method. High expression of Nrf2-, as well as glucocorticoid-dependent genes, were seen using the 3D culture method, while 2D hepatocytes exhibited rapid decline, followed by consistent low expression of both gene sets21.
3D Cell Culture Models
A wide variety of techniques currently exist to culture cells into 3D structures. These can be grouped into two main
categories; scaffold and non-scaffold based, and include the following individual technologies:
Polymeric Hard Scaffolds
Biologic Scaffolds
Micropatterned Surface Microplates
Non-Scaffold Based
Hanging Drop Microplates
Spheroid Microplates containing Ultra-Low Attachment (ULA) coating
Microfluidic 3D Cell Culture
Scaffold Based 3D Cell Culture
Polymeric Hard Scaffolds
3D tumor and tissue models can be created by culturing cells on pre-fabricated scaffolds, or matrices, designed to mimic the in vivo ECM. Cells attach, migrate, and fill the interstices within the scaffold to form 3D cultures22. The
scaffolds are used as a physical support system for in vitro cell culture, and have also shown promise for use with in
vivo tissue regeneration, as they have the potential to recreate the natural physical and structural environment of living
tissue23. Multiple geometric configurations of the commonly incorporated polymers, including polystyrene (PS) and polycaprolactone (PCL), exist. As seen in Figure 3, these include porous disc (3A), electrospun (3B), and orthogonal layering (3C).
Figure 3. (A) Porous disc (Image courtesy of James Weaver and Mooney lab, HSEAS and Wyss Institute); (B)
Electrospun (Image courtesy of The Electrospinning Company, Ltd.); and (C) Orthogonal layering (Image courtesy of 3D
Biotek, LLC) geometric configurations of polymeric 3D scaffolds.
Polymeric hard scaffolds are incorporated into two distinct research areas; regenerative medicine and preclinical in vitro testing. In the former, cells are grown on the scaffold with the goal of eventual in vivo transplantation to replace degenerative or altered tissue. Currently, scaffolds are used for engineering bone, cartilage, ligament, skin, vascular, neural, and skeletal muscle tissues24. Ge et al., in particular, developed a 3D printing method using a lactide and glycolide copolymer which supported proliferation and osteogenic differentiation of osteoblasts25. Evaluation of bone regeneration efficacy demonstrated that new bone tissue formation and maturation occurred within the scaffold over a 24 week period.
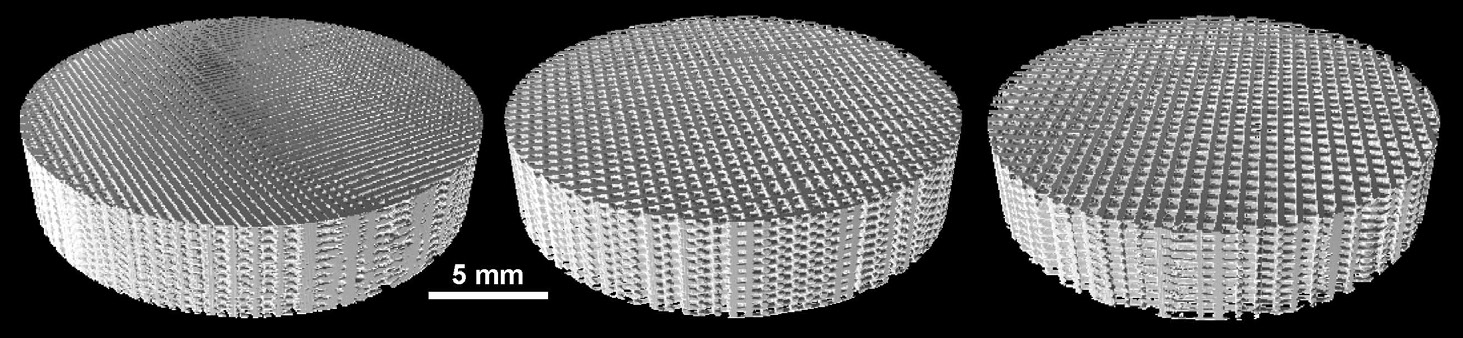
White Paper
For preclinical in vitro testing, cells are grown on the scaffold for the sole purpose of modeling tumors or tissue in a laboratory setting. Once the scaffolds are formed, they are cut to a diameter that fits into the appropriate test vessel; typically Petri dishes, or a microplate well (Figure 4). Typical thickness of the final scaffold is 150-200 µm; each containing consistent pore size.
Figure 4. Preparation of Orthogonal Layering polymeric 3D scaffolds for insertion into test vessel
(Image courtesy of NIST).
Once scaffolds are inserted (Figure 5), cell treatment and assay component dispensing procedures are then performed in a manner akin to that used with 2D cell culture. The arrangement of fibers and pores allow cells to remain close to nutrient sources, enabling exchange of nutrients, waste material, and gases similar to that seen in vivo. Using this knowledge, Bergenstock et al. illustrated that MCF-7 and HepG2 cancer cell lines, cultured in 3D using PS scaffolds as well as in a traditional 2D format, exhibited greater levels of proliferation and metabolic activity, as witnessed by higher A absorbance values across incubation periods up to two weeks. A reduced cytotoxic effect from treatment with the
anticancer drugs tamoxifen and methotrexate was also seen during the same timeframe from 3D cultured cells26.
Figure 5. Final joint configuration of polymeric 3D
scaffold in microplate format (Image courtesy of
The Electrospinning Company, Ltd.)
Biological Scaffolds
Scaffolds can also be created from components of a more natural or biological origin, such as proteins commonly found in the in vivo ECM. These commonly include, but are not limited to, fibronectin, collagen, laminin, and gelatin. Biological scaffolds not only provide a matrix to which the cells can attach and reorganize into 3D structures, but more importantly, they provide the correct microenvironment of soluble growth factors, hormones, and other molecules that cells interact with in an in vivo environment, which can alter gene and protein expression27. Current methods call for cells to either be mixed with scaffold proteins in a liquid state (hydrogel) prior to plating in a microplate well (Figure 6A), or added to previously formed scaffolds, or to have the protein mixture overlaid onto cells already aggregated into 3D spheroids. Cells can then restructure the surrounding environment to release signaling molecules, allow migration, or accommodate other cellular functions (Figure 6B). The end result is the creation of a proper homeostatic state.
White Paper
Figure 6. (A) Representation of collagen fibers suspended in medium following dispensing into a microplate well.
(Image courtesy of Lonza, Inc.); (B) HT-1080 cell invasion into a 3D collagen matrix 28.
Since these hydrogels are derived from natural sources, they promote many cellular functions, leading to increased viability, and proliferation of numerous cell types. They can also be advantageous to use over polymeric scaffolds, as the latter lack endogenous factors that promote appropriate cell behavior and act mainly to permit cell function29.
Hydrogels also offer the caveat of using multi-layer formats to form tissue-like structures. Individual cell types are embedded into separate hydrogel suspensions and layered on top of each other. Cells then organize within the hydrogel to form the layers found within in vivo tissues. Permeable supports can also be incorporated to simulate various air-liquid interfaces in an in vitro manner. Examples include bilayers resembling the dermis and epidermis of human skin30 and human corneal limbal crypts31.
Micropatterned Surface Microplates
Micropatterned surface plates take advantage of recent progress made in micro-fabrication technology. Each plate contains micrometer sized compartments, regularly arrayed on the bottom of each well. Wells can be various shapes, including square, round, or square with slits between the barriers of adjacent wells (Figure 7A-C). The different configurations are optimized for either spheroid or cell networking formation depending on the cell type being used.
Figure 7. Micropatterned microplates containing a (A) round, (B) square, or (C) slit patterning within the plate well (Images courtesy
of Kuraray Co., Ltd.)
Wells are coated to create a low adhesion surface within each micro-space. In this way, cells added to the well initially attach to the bottom of the micro-space, then aggregate together to form spheroid-like structures in the compartment over subsequent days of culture. Or in the case of the slit pattern, form contiguous cell networks along the bottom of the well (Figure 8).
Figure 8. Slit-type micropatterned microplates
containing cell network (Image courtesy of
Kuraray Co., Ltd.)
White Paper
The bottom of the plate is made of a thin, transparent film that is suitable for microscopic imaging of the cellular structures. Recent studies confirm that cells cultured using micropatterned plates exhibit different enzyme expression levels and drug reactivity compared to culturing in traditional 2D format. Kobayashi et al.demonstrated that the hepatocellular carcinoma cell line, FLC-4, exhibited increased expression levels of drug metabolizing enzymes, including CYP3A4, CYP2C9 and UGT1A1 when cultured on micropatterned plates in comparison to the same cells cultured in traditional 2D format32 . Results were consistent with rat primary hepatocytes cultured as spheroids, confirming that the morphological change and cell-cell interactions were the cause of the increased enzyme expression33.
Non-Scaffold Based 3D Cell Culture
Hanging Drop Microplates
Hanging drop plates (HDP) take advantage of the fact that cells, in the absence of a surface with which to attach, will self assemble into a 3D spheroid structure. Each plate conforms to SBS standards, but instead of containing normal wells with a traditional bottom, HDP well bottoms contain an opening. The top of HDP wells resemble a conventional microplate where cells in media can be dispensed (Figure 9A), while the aperture of the bottom opening is carefully designed to form a discrete droplet of media sufficient for cellular aggregation, but also small enough that surface tension prevents the droplets from being dislodged during manipulation (Figure 9B). Cells in the suspended media droplet aggregate over the course of hours to days creating the final spheroid structure.
Figure 9. Visualization of HDP top and bottom openings (Images courtesy of 3D Biomatrix).
Spheroid size is controlled by the number of cells dispensed into each drop. Co-cultured spheroids can also be created by adding multiple cell types either at the time of initial dispensing, or sequentially, allowing each set of cells to aggregate into separate layers.
For long-term culturing of spheroids, and to conduct assays, spheroids are typically transferred from the hanging drop plate to a second plate capable of containing larger media or buffer volumes (Figure 10). The larger volume ensures that the aggregated cells are in the presence of suitable conditions, such as nutrient levels and pH, for propagation periods reaching days or even weeks.
Figure 10. Hanging drop spheroid aggregation plate
and secondary assay/propagation plate. (Images
courtesy of 3D Biomatrix).
White Paper
Due to the round configuration of the 3D cellular structure, spheroids are well-suited for use as primary cell-based tissue models and tumor models that include immortalized cancer cell lines. This is confirmed by work performed by Kermanizadeh, et al. using a 3D human liver microtissue model to examine potential chronic hepatotoxic effects of nanomaterials34. Ovarian cancer spheroids, created using a 384-well hanging drop platform, were also used incorporated by Raghavan, et al. for use with preclinical drug sensitivity assays35. Spheroids formed using hanging drop methods can also be embedded into biological scaffolds to mimic the ECM surrounding cancerous tumors. The combination allows for an in vivo-like examination of tumor metastasis using an in vitro 3D model36.
Spheroid Microplates containing Ultra-Low Attachment (ULA) coating
Spheroid microplates can also be incorporated to create the same round multi-cell tissue or tumor models generated using hanging drop plates. Plates have the typical well shape and depth of an SBS 96- or 384-well microplate. Because of the larger media and reagent volume capacity within the well, spheroid aggregation, propagation, and experimental procedures can be carried out in the same plate; without the need for transfer to a second microplate.
Figure 11. (A) Clear (Image courtesy of PerkinElmer, Inc.) and (B) black walled, clear bottom ULA
spheroid microplates in 96- and 384-well configurations (Image courtesy of Corning, Inc.)
The ULA surface coating added to the well bottom minimizes cell adherence to allow spheroid formation. Well bottoms also possess either a round, tapered (Figure 12) or v-shaped geometry that both ensures the creation of consistent, single spheroids and also helps to position the spheroids in the middle of the well.
Figure 12. ULA spheroid microplates possessing (A) round (Image courtesy of Corning, Inc.) and (B)
tapered well bottom configurations (Image courtesy of InSphero).
ULA spheroid microplate-based cell aggregation and assay performance is also incorporated into current research methods. Ivanov, et al. demonstrated how neural stem cells could be added to ULA spheroid microplates. Aggregation then took place creating 3D neurospheres that were used to monitor growth kinetics and drug toxicity over time37. Similar work was also performed by Vinci, et al. who tested a variety of immortalized cell lines to make tumor spheroids, or tumoroids, in spheroid microplates38. Propagation and cell viability after exposure to cytotoxic agents was once again examined over a multi-day incubation period. Testing then extended to the addition of an ECM to previously formed spheroids, such that the spheroid was embedded completely in the matrix. Experiments then confirmed that, using this configuration, 3D tumor invasion assays could be performed with spheroid microplates.
White Paper
Microfluidic 3D Cell Culture
As previously mentioned, 3D cell culture techniques strive to recreate the three-dimensional architecture of in vivo tissues and tumors, as well as the interactions between cells and ECM. Microfluidic platforms can also be used to create similar heterogeneous models, while contributing an additional level of complexity by introducing a perfusive flow aspect to the cellular environment; allowing for continuous nutrition and oxygen introduction as well as waste removal through culture medium. Cells are maintained within a compartment by disparate physical or non-physical barriers. Media containing nutrients, chemicals, treatment molecules, or staining reagents is then perfused past the cells (Figure 13). Predefined gaps in the barriers allow interactions between compartments.
Figure 13. Representation of perfusion in a microfluidic 3D culture system. Cells are maintained
in a predefined compartment by micropillars, allowing interplay with perfused media (Image
courtesy of EMD Millipore Corporation).
Physical barriers incorporated into microfluidic devices are traditionally composed of glass or silicon, polymers including polydimethylsiloxane (PDMS), polymethylmethacrylate (PMMA), polycarbonate (PC) and polystyrene (PS), in addition to chromatographic or filter paper39. Cells can also be combined with a supporting matrix, such as a collagen or Matrigel®, to encourage cell-ECM interaction and encourage assembly into 3D structures. Introduction of an ECM also allows for the creation of microfluidic devices that do not incorporate physical barriers. Matrix polymerization maintains the cells in the pre-defined culture area and also acts as a filter during the perfusive flow40.
Microfluidic systems are also used for varied 3D cell culture applications including stem, primary, and cancer cells. Yu, et al. developed a 3D microfluidic cell culture system and applied this system to the study of the differentiation of rat bone marrow mesenchymal stem cells (BMSCs) in vitro41. A PDMS device was also developed by Wan, et al. to investigate the differentiation of murine embryonic stem cells into cardiomyocytes42. Finally, Liu, et al. incorporated a microfluidic device to investigate the effect of carcinoma-associated fibroblasts on cancer cell invasion in a 3D matrix43.
The list of available 3D cell culture technologies can be daunting when trying to decide which is the most appropriate for a particular application. While all methods strive to create in vivo-like cellular environments, certain techniques are more amenable to re-creating in vitro barrier models (i.e. skin and lung). Others are more suitable to mimicking cancerous tumors or tissues. Traditional assays, developed for use with 2D cultured cells, may also require re-optimization due to the need for components to penetrate matrices and 3D cellular structures hundreds of microns in diameter. Unlabeled cellular imaging as an analysis technique should also be considered, as not all matrices are transparent, or plate/device design may limit accurate cellular visualization. Keeping these qualifications in mind, the correct 3D cell culture technique can be incorporated to meet the desired research goal.
White Paper
1. Kola, I.; Landis, J. Can the pharmaceutical industry reduce attrition rates? Nat. Rev. Drug Discov. 2004, 3 (8), 711-715.
2. Knight, A. Systematic reviews of animal experiments demonstrate poor human clinical and toxicological utility.
ATLA-Altern. Lab Anim. 2007, 35 (6), 641-659.
3. de Boo, J.; Hendriksen, C. Reduction strategies in animal research: a review of scientific approaches at the intra-
experimental, supra-experimental and extra-experimental levels. ATLA-Altern. Lab Anim. 2005, 33 (4), 369-377.
4. Mazzoleni, G.; Di Lorenzo, D.; Steimberg, N. Modeling tissues in 3D: the next future of pharmaco-toxicology and
food research? Genes Nutr. 2009, 4 (1), 13-22.
5. Lovitt, C. J.; Shelper, T. B.; Avery, V. M. Advanced cell culture techniques for cancer drug discovery. Biology 2014, 3
(2), 345-367.
6. Jain, R. K.; Forbes, N. Can engineered bacteria help control cancer? PNAS. 2001, 28 (26), 14748-14750.
7. Gurski, L. A.; Petrelli, N. J.; Jia, X.; Farach-Carson, M.C. 3D matrices for anti-cancer drug testing and development.
Oncol. Issues 2010, 25, 20-25.
8. Ghosh, S.; Spagnoli, G. C.; Martin, I.; Ploegert, S.; Demougin, P.; Heberer, M.; Reschner, A. Three-dimensional
culture of melanoma cells profoundly affects gene expression profile: a high density oligonucleotide array study. J. Cell Physiol. 2005, 204 (2), 522-531.
9. Holmes, A. M.; Creton, S.; Chapman, K. Working in partnership to advance the 3Rs in toxicity testing. Toxicology
2010, 267 (1-3), 14-19.
10. National Research Council. Toxicity Testing in the 21st Century: A Vision and a Strategy. Washington, DC: The
National Academy Press, 2007.
11. Marion, M. J.; Hantz, O.; Durantel, D. The HepaRG cell line: biological properties and relevance as a tool for cell
biology, drug metabolism, and virology studies. Methods Mol Biol. 2010, 640, 261-272.
12. LeCluyse, E. L.; Bullock, P. L.; Parkinson, A. Strategies for restoration and maintenance of normal hepatic structure
and function in long-term cultures of rat hepatocytes. Adv. Drug Deliver. Rev. 1996, 22 (1), 133-186.
13. Vinci, M.; Gowan, S.; Boxall, F.; Patterson, L.; Zimmermann, M.; Court, W.; Lomas, C.; Mendiola, M.; Hardisson, D.;
Eccles, S. A. Advances in establishment and analysis of three-dimensional tumor spheroid-based functional assays for target validation and drug evaluation. BMC Biol. 2012, 10, 29.
14. Dhiman, H. K.; Ray, A. R.; Panda, A. K. Three-dimensional chitosan scaffold-based MCF-7 cell culture for the
determination of the cytotoxicity of tamoxifen. Biomaterials 2005, 26 (9), 979-986.
15. Howes, A. L.; Chiang, G. G.; Lang, E. S.; Ho, C. B.; Powis, G.; Vuori, K.; Abraham, R. T. The phosphatidylinositol
3-kinase inhibitor, PX-866, is a potent inhibitor of cancer cell motility and growth in three-dimensional cultures. Mol. Cancer Ther. 2007, 6 (9), 2505-2514.
16. Lee, G. Y.; Kenny, P. A.; Lee, E. H.; Bissell, M. J. Three-dimensional culture models of normal and malignant breast
epithelial cells. Nat. Methods 2007, 4 (4), 359-365.
17. Bazou, D. Biochemical properties of encapsulated high-density 3-D HepG2 aggregates formed in an ultrasound
trap for application in hepatotoxicity studies: Biochemical responses of encapsulated 3-D HepG2 aggregates. Cell Biol. Toxicol. 2010, 26 (2), 127-141.
18. Erickson, I. E.; Huang, A. H.; Chung, C.; Li, R. T.; Burdick, J. A.; Mauck, R. L. Differential maturation and structure-
function relationships in mesenchymal stem cell- and chondrocyte-seeded hydrogels. Tissue Eng. Part A 2009, 15 (5), 1041-1052.
19. Wu, F. J.; Friend, J. R.; Hsiao, C. C.; Zilliox, M. J.; Ko, W. J.; Cerra, F. B.; Hu, W. S. Efficient assembly of rat hepatocyte
spheroids for tissue engineering applications. Biotechnol. Bioeng. 1996, 50 (4), 404-415.
20. Nemoto, N.; Sakurai, J. Activation of Cyp1a1 and Cyp1a2 genes in adult mouse hepatocytes in primary culture. Jpn.
J. Cancer Res. 1993, 84 (3), 272-278.
21. Kratschmar, D. V.; Messner, S.; Moritz, W.; Odermatt, A. Characterization of a rat multi-cell type 3D-liver microtissue
system. J. Tissue Sci. Eng. 2013, 4 (2).
22. Sourla, A.; Doillon, C.; Koutsilieris, M. Three dimensional type I collagen gel system containing MG-63 osteoblasts-
like cells as a model for studying local bone reaction caused by metastatic cancer cells. Anticancer Res. 1996, 16 (5A), 2773-2780.
23. Tan, W.; Krishnaraj, R.; Desai, T. A. Evaluation of nanostructured composite collagen-chitosan matrices for tissue
engineering. Tissue Eng. 2001, 7 (2), 203-210.
24. Dhandayuthapani, B.; Yoshida, Y.; Maekawa, T.; Kumar, D. S. Polymeric scaffolds in tissue engineering application: A
review. Int. J. Polym. Sci. 2011, 2011.
25. Ge, Z.; Tian, X.; Heng, B. C.; Fan, V.; Yeo, J. F.; Cao, T. Histological evaluation of osteogenesis of 3D-printed poly-
lactic-co-glycolic acid (PLGA) scaffolds in a rabbit model. Biomed. Mater. [Online] 2009, 4 (2), 021001, http://iopscience.iop.org/article/10.1088/1748-6041/4/2/021001/pdf (accessed Sep 21, 2015).
White Paper
26. Bergenstock, M. K.; Balaburski, G. M.; Lau, W.; Sun, W.; Sun, Q.; Liu, Q. Polystyrene scaffolds: A novel tool for in
vitro three-dimensional cancer models. Presented at the First AACR International Conference on Frontiers in Basic Cancer Research, October 8-11, 2009.
27. Birgersdotter, A.; Sandberg, R.; Ernberg, I. Gene expression perturbation in vitro-A growing case for three-
dimensional (3D) culture systems. Semin. Cancer Biol. 2005, 15 (5), 405-412.
28. Fisher, K. E.; Pop, A.; Koh, W.; Anthis, N. J.; Saunders, W. B.; Davis, G. E. Tumor cell invasion of collagen matrices
requires coordinate lipid agonist-induced G-protein and membrane-type matrix metalloproteinase-1-dependent signaling. Mol Cancer. 2006, 5 (69).
29. Cushing, M. C.; Anseth, K. S. Materials science. Hydrogel cell cultures. Science, 2007, 316 (5828), 1133-134.
30. Torkian, B. A.; Yeh, A. T.; Engel, R.; Sun, C. H.; Tromberg, B. J.; Wong, B. J. Modeling aberrant wound healing using
tissue-engineered skin constructs and multiphoton microscopy. Arch Facial Plast Surg, 2004, 6 (3), 180-187.
31. Levis, H. J.; Massie, I.; Dziasko, M. A.; Kaasi, A.; Daniels, J. T. Rapid tissue engineering of biomimetic human corneal
limbal crypts with 3D niche architecture. Biomaterials, [Online] 2013, 34 (35), 8860-8868 http://www.sciencedirect.
com/science/article/pii/S0142961213009332 (accessed Sep 21, 2015).
32. Kobayashi, K.; Yoshida, A.; Ejiri, Y.; Takagi, S.; Mimura, H.; Hosoda, M.; Matsuura, T.; Chiba, K. Increased expression
of drug-metabolizing enzymes in human hepatocarcinoma FLC-4 cells cultured on micro-space cell culture plates. Drug Metab Pharmacokinet. [Online] 2012, 27 (5), 478-485 https://www.jstage.jst.go.jp/article/dmpk/27/5/27_DMPK-12-RG-016/_pdf (accessed Sep 21, 2015).
33. Hamamoto, R.; Yamada, K.; Kamihira, M.; Iijima, S. Differentiation and proliferation of primary rat hepatocytes
cultured as spheroids. J Biochem. 1998, 124 (5), 972-979.
34. Kermanizadeh, A.; Løhr, M.; Roursgaard, M.; Messner, S.; Gunness, P.; Kelm, J. M.; Møller, P.; Stone, V.; Loft, S.
Hepatic toxicology following single and multiple exposure of engineered nanomaterials utilising a novel primary human 3D liver microtissue model. Part Fibre Toxicol. 2014, 11 (56).
35. Raghavan, S.; Ward, M. R.; Rowley, K. R.; Wold, R. M.; Takayama, S.; Buckanovich, R. J.; Mehta, G. Formation of
stable small cell number three-dimensional ovarian cancer spheroids using hanging drop arrays for preclinical drug sensitivity assays. Gynecol Oncol. [Online] 2015, 138, 181-189 http://www.gynecologiconcology-online.net/article/S0090-8258(15)00833-1/fulltext (accessed Sep 21, 2015).
36. Guzman, A.; Ziperstein, M. J.; Kaufman, L. J. The effect of fibrillar matrix architecture on tumor cell invasion of
physically challenging environments. Biomaterials. [Online] 2014, 35 (25), 6954-6963 http://www.sciencedirect.com/science/article/pii/S0142961214004840 (accessed Sep 21, 2015).
37. Ivanov, D. P.; Parker, T. L.; Walker, D. A.; Alexander, C.; Ashford, M. B.; Gellert, P. R.; Garnett, M. C. Multiplexing
spheroid volume, resazurin and acid phosphatase viability assays for high-throughput screening of tumour spheroids and stem cell neurospheres. PLoS One. [Online] 2014, 9 (8), e103817 http://journals.plos.org/plosone/article?id=10.1371/journal.pone.0103817 (accessed Sep 23, 2015.
38. Vinci, M.; Gowan, S.; Boxall, F.; Patterson, L.; Zimmerman, M.; Court, W.; Lomas, C.; Mendiola, M.; Hardisson, D.;
Eccles, S. A. Advances in establishment and analysis of three-dimensional tumor spheroid-based functional assays for target validation and drug evaluation. BMC Biol. [Online] 2012, 10, 29 http://www.ncbi.nlm.nih.gov/pmc/articles/PMC3349530/ (accessed Sep 23, 2015).
39. Li, X. J.; Valadez, A. V.; Zuo, P.; Nie, Z. Microfluidic 3D cell culture: potential application for tissue-based bioassays.
Bioanalysis. 2012, 4 (12), 1509-1525.
40. Jang, M.; Neuzil, P.; Volk, T.; Manz, A.; Kleber, A. On-chip three-dimensional cell culture in phaseguides improves
hepatocyte functions in vitro. Biomicrofluidics. [Online] 2015, 9 (3), 034113, http://scitation.aip.org/content/aip/journal/bmf/9/3/10.1063/1.4922863 (accessed Sep 23, 2015).
41. Toh, Y. C.; Zhang, C.; Zhang, J.; Khong, Y. M.; Chang, S.; Samper, V. D.; van Noort, D.; Hutmacher, D. W.; Yu, H. A
novel 3D mammalian cell perfusion-culture system in microfluidic channels. Lab Chip. [Online] 2007, 7 (3), 302-209 http://pubs.rsc.org/en/Content/ArticleLanding/2007/LC/b614872g#!divAbstract (accessed Sep 23, 2015).
42. Wan, C. R.; Chung, S.; Kamm, R. D. Differentiation of embryonic stem cells into cardiomyocytes in a compliant
microfluidic system. Ann Biomed Eng. [Online] 2011, 39 (6), 1840-1847 http://link.springer.com/article/10.1007%2Fs10439-011-0275-8 (accessed Sep 23, 2015).
43. Liu, T.; Lin, B.; Qin, J. Carcinoma-associated fibroblasts promoted tumor spheroid invasion on a microfluidic 3D co-
culture device. Lab Chip. [Online] 2010, 10 (13), 1671-1677 http://pubs.rsc.org/en/Content/ArticleLanding/2010/LC/c000022a#!divAbstract (accessed Sep 23, 2015).
Source: http://www.biotekinstruments.com.br/assets/tech_resources/3D%20Cell%20Culture%20White%20Paper_FINAL.pdf
MINISTÈRE DES AFFAIRES ÉTRANGÈRES & LE COMITÉ D'INFORMATION MÉDICALE (Ministère des Affaires Étrangères – Maison des Français à l'Étranger) Mémento à l'usage des professionnels de santé La Société de Médecine des VoyagesetLe Comité d'Information MédicaleMinistère des Affaires Étrangères –Maison des Français de l'Étranger EXPATRIATION ET SANTÉ,Mémento à l'usage des professionnels de santé
EMPRESA DE DESARROLLO URBANO DE BOLÍVAR S.A. NIT: 890.481.123-1 NOTAS A LOS ESTADOS FINANCIEROS A JUNIO 30 DE 2015 1. NOTAS DE CARÁCTER GENERAL 1. NATURALEZA JURIDICA, OBJETO SOCIAL ACTIVIDADES QUE DESAROLLA O COMETIDO ESTATAL. NATURALEZ-A JURIDICA La Empresa de Desarrollo Urbano de Bolívar -EDURBE S.A., es una Empresa Industrial y Comercial del estado, del Orden Distrital, constituida el 24 de Diciembre de 1981, mediante Escritura Número 2069 de la Notaria 2da de Cartagena, su capital es netamente público y sus